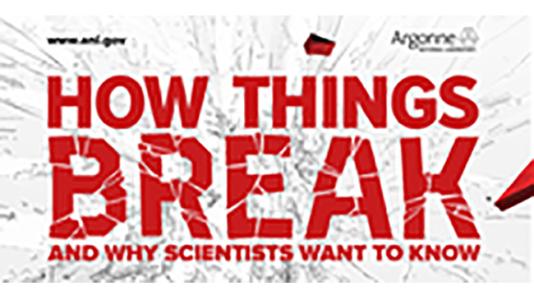
This article was originally published in the spring 2016 issue of Argonne Now, the laboratory’s science magazine.
Humans spend a lot of time creating things—this drives a huge amount of our lives, economically and personally—and we are always in a fight to keep them from breaking down. Houses, roads, cars. Power lines and bridges. Solar cells and computers. Batteries. People.
Then there are the things we want to break down, and are always searching for better ways to do it: Harmful pollutants in the soil. Old buildings. The cellulose in plant fibers, so we can make it into biofuels. Atoms, so we can harness the energy they release as nuclear energy, and find out what makes up the universe.
Much of our lives revolve around either of these categories, and they constantly occupy the minds of scientists and engineers. An entire lab at Argonne is devoted to finding out what goes wrong when batteries stop working. No fewer than five accelerators designed to smash tiny things into one another are running at any given time on the campus.
You break things when you want to know what they’re made of, and this is useful for answering both the most fundamental questions — like what the universe is made out of — and the most everyday questions, like why your cell phone battery dies after just a few hours.
To fix a performance problem, researchers want to know what exactly is happening when a device—the battery, a solar cell, an engine — doesn’t do what it’s supposed to do anymore. If you want to fix the problem, you need to know how it happens.
But finding out what’s happening at the molecular level is surprisingly, or maybe unsurprisingly, difficult to do. You can’t see atoms except in a very small cross-section, in a very thin sample, with very expensive and bulky instruments (an electron microscope, in case you’re wondering.) And real life is a lot messier than that. Lithium-ion batteries, for example, the kind in your cell phone and laptop and possibly your car, are made up of layers and layers of complex molecular machinery with both liquids and solids.
Much of science, in fact, is devoted to finding clever ways to intuit what atoms are doing based on clues they leave behind—the effects they have on things around them. Besides electron microscopes, there are many different types of instruments, each with its own strengths.
One of the most powerful instruments in the world for exploring tiny things is called the Advanced Photon Source, a Department of Energy user facility at Argonne. It’s an X-ray synchrotron, so big around a baseball stadium could sit inside its circular ring. Researchers aim extremely powerful X-ray beams at a sample of what they’re studying. Once a beam hits the sample and scatters, scientists can piece together information about the sample’s molecular and atomic structure.
At any given day at Argonne, scientists and engineers are breaking and building things all around the laboratory using dozens of different methods.
Another very powerful way to simulate how things might unfold in the real world is to build a computer program to model how it happens. This is very useful in industry, where companies can simulate a hundred different possible versions of their product to narrow it down to a handful of the very best prototypes to actually build in the lab. Supercomputers and scientists like those at the Argonne Leadership Computing Facility can build and run these models.
At any given day at Argonne, scientists and engineers are breaking and building things all around the laboratory using dozens of different methods.
We’ll follow along as scientists break three things: a battery, an atom, and a nuclear reactor.
BATTERIES
Deep in the Argonne battery-testing facility, rows of humming black towers hold five battery prototypes each. They are charging and draining the batteries over and over to find out how long each lasts before breaking down. Nearby, other machines are cooling and heating batteries to find out how temperature affects their performance.
Established in 1976, the computer-controlled lab has been running 24 hours a day ever since, testing prototype and production batteries from both private and government-funded initiatives. Over 100 batteries can be tested simultaneously in the lab—useful because a thorough testing can take from two months to two years.
“For example, we answer questions such as ‘What will happen to my battery if I fly out and leave my car in the airport parking lot for a week in January?’ ” said manager Ira Bloom, who runs the facility. “Or conversely, July?”
When the batteries have completed their rounds, they go back to developers along with the results of each test. By seeing how the batteries failed—or succeeded—the developers can change the battery’s design to improve performance and life.
But if you want to design an entirely new battery from scratch — as scientists are doing elsewhere at Argonne — you have to start further back.
There are hundreds of potential chemistries for the three main parts that make up a battery. These are the two electrodes, the positive cathode and negative anode, and the medium that lets lithium atoms swim back and forth between them, the electrolyte.
When scientists have an arrangement that looks promising, they cycle the battery at high temperatures to mimic what happens during years of use, said Lynn Trahey, a materials scientist with the Joint Center for Energy Storage Research, a Department of Energy Innovation Hub led by Argonne.
“High temperatures accelerate the unwanted side reactions that eventually reduce the battery’s performance,” she said. “The electrolyte starts to break down, and you start to see changes along the surfaces where it meets the electrodes.”
All the while, they’re measuring how long the battery still holds a charge, how much power it can put out. Then, when the cell finally dies, they open it up to do a post-mortem.
“All the side reactions change the physical landscape inside a cell,” Trahey said. “For example, some of the lithium might have formed dendrites that act like microscopic metal spikes inside of your battery, poking holes through the electrolyte and separator and letting the electrodes touch each other, which short-circuits the battery.”
Researchers can do all this in a special facility at Argonne called the Battery Post-Test Facility, one of the few facilities in the world capable of this kind of research. Inside a giant glovebox filled with inert gas, they can dismantle the battery, analyze it, and take images with an electron microscope to see that physical landscape.
These and other facilities at Argonne help scientists understand how different chemistries work together on the atomic and molecular level. This background helps scientists design safer, better, and cheaper batteries, which could make your cell phone stay charged longer—and also improve the driving range of electric cars, help our power grid keep electricity flowing smoothly, and make wind and solar easier to use by storing the electricity they generate.
ATOMS
“When we smash particles, we’re trying to find out what is out there.”
–Marcel Demarteau, High Energy Physics director
Atoms don’t break very easily (thank goodness, since they’re what you and everything you love is made of). So to break them, and therefore find out what they’re made of and what holds the universe together, you have to build an accelerator.
Accelerators take beams of particles and shoot them at nearly the speed of light until they smash—either into a target, or into one another. “Then you see what comes out,” said Marcel Demarteau, who leads Argonne’s High Energy Physics division.
At the heart of any atom is the nucleus. There’s a lot about nuclei we still don’t know; locked inside are the keys to understanding the forces at play in the cosmos and the story of how all of the elements were formed in stars and scattered across the universe.
At the Argonne Tandem Linac Accelerator System (ATLAS), scientists shoot beams of heavy nuclei at different targets. “By calibrating the energy at which the nuclei collide, we can fuse the nuclei, break them apart, or have them rotate together, picking up pieces of the target,” said Robert Janssens, director of the Physics division at Argonne. Each interaction creates data that illuminate different pieces of the interactions that govern matter.
Physicists from more than 90 U.S. institutions and 18 countries have conducted experiments at the Argonne Tandem Linac Accelerator System since it was completed in 1985.
If you want to go smaller, into the parts that make up the parts of the atom, you are delving into a realm of physics called high-energy physics—and you need an extremely powerful accelerator, like the Large Hadron Collider in Switzerland.
In high-energy physics, scientists put two different types of particles into accelerators: protons (or anti-protons, their antimatter counterparts) and electrons (or their antimatter counterpart, the positron).
Protons are made up of several sub-particles. “The way it’s often explained is that smashing protons is like throwing two garbage cans at each other. A whole bunch of stuff comes out,” Demarteau said. These are quarks and the things that hold quarks together, called gluons.
Fundamentally helpful
The study of antimatter and ways to detect it led directly to the development of the PET scan. (The P in PET is positron, the antimatter counterpart of an electron). “Fifty years after antimatter was theoretically proposed, this technology was in every hospital around the country,” Demarteau said.
But an electron is a “fundamental” particle, which means it has no substructure. So when electrons and positrons collide, the collisions are much cleaner; the particles annihilate in a flash of light and then rematerialize as entirely different particles.
“When we smash particles, we’re trying to find out what is out there,” Demarteau said. “What are the fundamental constituents of matter made out of? Are there forms of matter we don’t know about?”
And there are a lot of things we don’t know. A partial list of physics’ current greatest mysteries: Some huge amount of “dark matter” out in the galaxy is causing light to bend oddly around galaxies, but we can’t see it directly and don’t know where it came from. As you read this trillions of particles called neutrinos are zipping through your body, but they’re so difficult to catch or detect that we know there are different kinds, but not how much mass they have or which is the heaviest. And there’s a lot more matter than anti-matter out there, which doesn’t make sense according to the models we have.
The answers to all of these questions are locked inside particles, waiting to be broken open and teased apart in accelerators.
NUCLEAR REACTORS
When scientists were figuring out how to break an atom, they also discovered that breaking atoms can release energy. A lot of energy; one piece of uranium the size of a tennis ball could generate all the electricity you’ll use over the course of a lifetime.
When you split the nucleus of a heavy, unstable atom like uranium, it gives off a burst of energy, some of which is in the form of heat. Nuclear reactors capture this heat to boil water into steam. From then on it’s the same as a coal or natural gas plant; the steam turns a turbine that makes electricity.
Inside the heart of a reactor, the pressure, heat, and radiation are intense. Engineers designing the plant to withstand these conditions want to know exactly how the concrete, steel, and fuel rods react over the course of their lifetimes, which are typically several decades.
“Being inside a nuclear reactor changes the microstructure of a material,” said Argonne materials scientist Walid Mohamed. The microstructure is what the material looks like if you zoom in very close; it might have long thin rods, or a strict ordered lattice, or big interlocking puzzle pieces.
These different arrangements give the materials very different properties — some bend easily, others are stronger, others conduct heat or electricity well. But being subjected to the harsh environment eventually changes the microstructure, and hence the properties. This is what engineers designing and maintaining reactors want to know.
“For example, nuclear fuels materials will swell and expand when irradiated, but we want to know where and how it will swell,” Mohamed said. Does it crack or form tiny bubbles (called voids)? How big? How quickly? Where?
“Because there’s steam inside a reactor, the materials will also be oxidizing,” Mohamed said. The surface of the metal will react with the steam to form a layer of oxide on top; engineers want to know exactly how thick that will be and how long it will take to form.
To find out, Mohamed and other researchers are using high-brightness, high-energy X-rays from the Advanced Photon Source to peer inside the metals. The APS allows them to gather microstructural data even as they are twisting, pulling, heating, and pressurizing the materials to form cracks and defects.
To simulate the effects of radiation—which tends to make things more brittle — they use ATLAS to shoot xenon ions at the test nuclear fuel material, then take it to the APS.
After you find out how the material performed, then you can modify it to make it do better. “For example, adding nanostructures to a material can make it stronger and resistant to damage, said Argonne nuclear engineer Meimei Li. “Radiation creates defects, but nanostructures increase the amount of surfaces inside a material. This lets it absorb defects, which improves its radiation resistance.”
“The fact that we can gather X-ray data in real time while these materials deform is extremely important,” said Jonathan Almer, an Argonne physicist who works with Li at the APS. “It gives scientists and engineers who used to have as little as one data point—i.e., how long it takes before this material breaks—now up to millions of them.”
There’s more to the science of “breaking” than physical objects like atoms and batteries. As climate change brings us more floods, more heat and drought, and higher sea levels, we’ll need to plan ahead to make sure our infrastructure doesn’t break — which is why Argonne has a community of researchers helping planners look ahead. Learn more at Weather Or Not We’re Ready.
They can take all their data to the Argonne Leadership Computing Facility, where they work with computational scientists to produce maps of the evolving materials with unprecedented detail. The data can then be compared to complex simulations of reactors also performed at the computing facility.
Together, these studies can help existing reactors safely extend their lifetimes, Li said, or model new, advanced reactor designs that are more efficient, create less waste, and operate more safely.
Research described in this story is conducted at the Advanced Photon Source, a U.S. Department of Energy Office of Science User Facility, at beamlines 1-ID and 32-ID, and is supported by the DOE Office of Science. The Battery Test Facility and Post-Test Facility are supported by the Vehicle Technologies Office at the DOE Office of Energy Efficiency & Renewable Energy. JCESR is a DOE Energy Innovation Hub funded by the Office of Science. Additional research takes place at the Argonne Tandem Linac Accelerator System and the Argonne Leadership Computing Facility, both DOE Office of Science User Facilities. Argonne and other national labs’ work at the Large Hadron Collider, including parts of the detector built at Argonne, is supported by the Office of Science and the National Science Foundation.
How Things Break (And Why Scientists Want to Know)
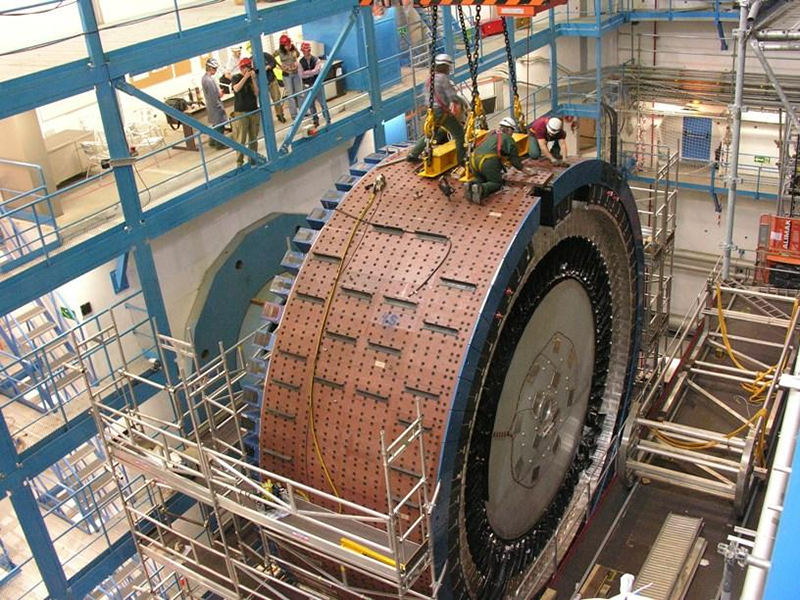
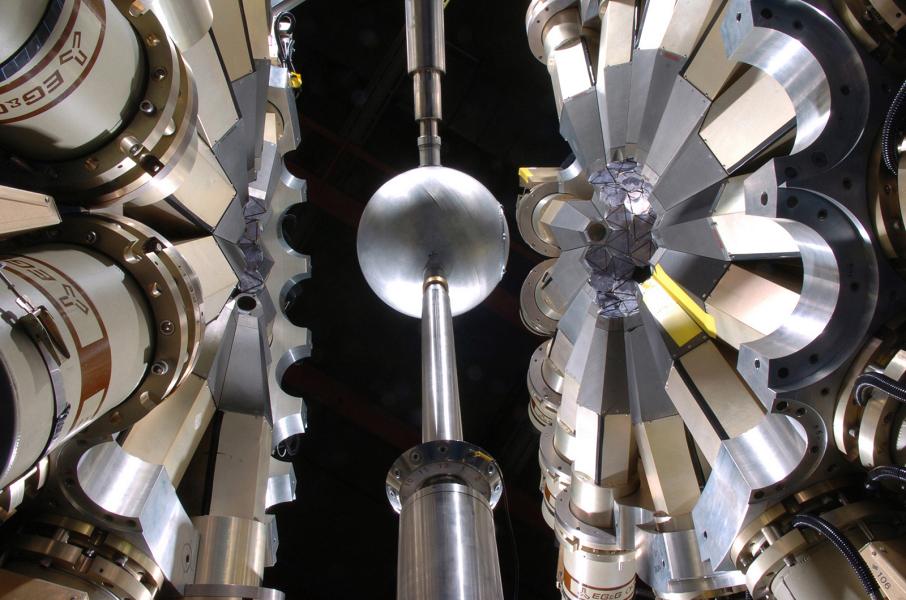
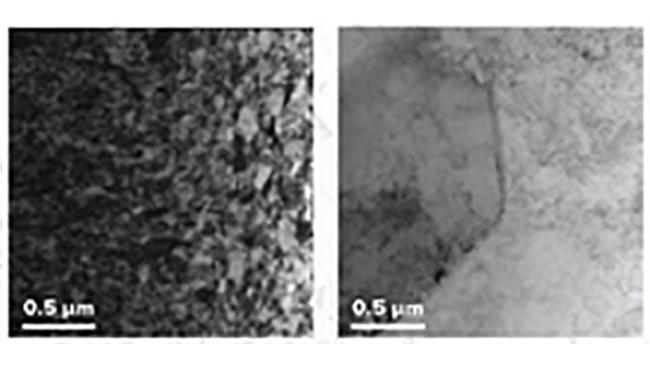